New insights into how the brain stores memories
November 26, 2012
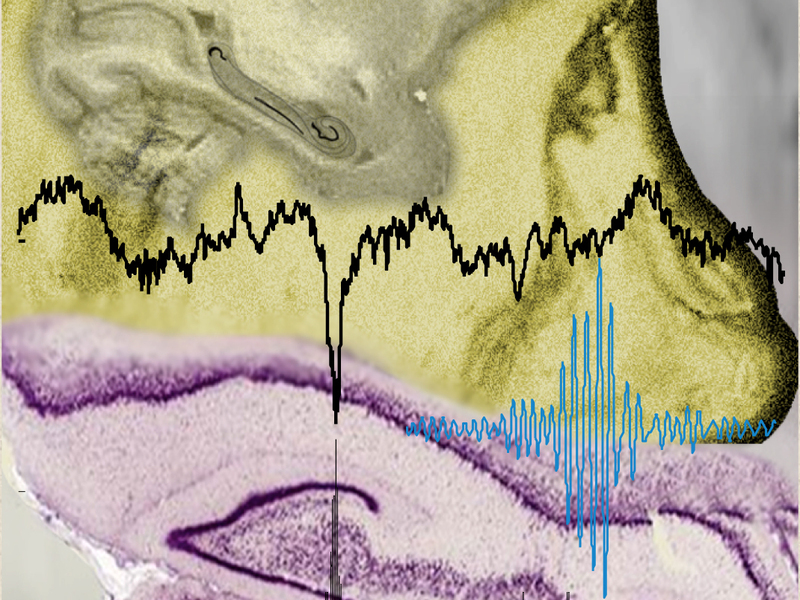
Oscillations in the hippocampus: neural interactions during non-REM sleep and periods of silence (credit: MPI for Biological Cybernetics)
Exactly how does long-term memory get updated (or “written,” in computer language)?
One hypothesis, for example, is that while in deep dreamless sleep, the hippocampus sends messages to the cortex and changes its plasticity, transferring recently acquired knowledge (in short-term memory) to long-term memory.
Background
Many invasive studies in nonhuman primates and clinical investigations in human patients have demonstrated that the hippocampus, one of the oldest, most primitive brain structures, is largely responsible for the long term retention of specific information regarding places, events, and their contexts, that is, for the retention of “declarative memories.”
Without a hippocampus, a person could learn a manual task over a period of days, say, playing a simple instrument, but — remarkably — such a skill is acquired in the absence of any memory of having practiced the task before.
The consolidation of declarative memory is thought to occur in two subsequent steps:
- In the encoding phase, the hippocampus rapidly binds (encodes) neocortical representations to local memory traces.
- During subsequent “off-line” periods of calmness or sleep, the new traces are concurrently reactivated in both the hippocampus and the cortex to strengthen the cortico-cortical connections underlying learned representations.
How does the hippocampus communicate with the cortex?
So what is the neural basis of this hippocampal-cortical dialog, and how does the hippocampus communicate with the rest of the brain?
To find out, scientists from the Max Planck Institute for Biological Cybernetics developed led by Nikos Logothetis, director of the Department for Physiology of Cognitive Processes, developed a “neural event-triggered functional magnetic resonance imaging” (NET-fMRI) method. It uses multiple-contact electrodes in combination with functional magnetic resonance imaging (fMRI) of the entire brain to map widespread networks of neurons that are activated by local, structure-specific neural events.
They wanted to find out exactly which brain areas consistently increased or decreased their activity in relationship to a certain type of fast hippocampal oscillations known as “ripples,” which occur primarily during deep sleep and can be measured with electrophysiological methods.
Suppressing interference

Examples of ripple-triggered maps of activation in awake monkeys. Widespread activations were found in hippocampal formation (HF), extrastriate occipital areas (XC), sensorimotor cortex (SM), temporal (Temp) and parietal (Par) cortical areas, as well as in the prefrontal cortex (PFC). In contrast, subcortical structures, including basal ganglia (BG), cerebellum (Cer), tectum and thalamus (Thal), as well as the primary visual cortex (V1), showed robust signal decreases. (Credit: MPI for Biological Cybernetics)
Using intracranial recordings of neural field potentials in both anesthetized and awake, behaving monkeys, the scientists got the answer: the short periods of aperiodic (irregular), recurrent high-frequency ripples (which occur during sleep and calmness) are closely associated with reproducible cortical activations that occur at the same time as extensive activity suppression in other brain structures. These structures are in fact the ones whose activities could, in principle, interfere with the hippocampal-cortical dialog.
So during off-line memory consolidation, synergistic thalamocortical activity may be orchestrating a privileged interaction state between hippocampus and cortex by silencing the output of subcortical centres involved in sensory processing or potentially mediating procedural learning.
In other words, the ripples created a clear channel to avoid interference with cortical memory recordings. (An analogy would be a cable that’s shielded from other signals.)
They found that the suppression of activity in the thalamus, for instance, reduces signals related to sensory processing, while the suppression of the basal ganglia and the cerebellar cortex may reduce signals related to other memory systems, such as that underlying procedural learning, for example riding a bicycle.
The researchers say this study offers new insights into the large-scale organization of memory — a cognitive capacity emerging from the activation of widespread neural networks that were impossible to study in depth before now, using either functional imaging alone or traditional single neuron recordings.
The combined method is important because the vast majority of neurological failures reflect dysfunctions of large-scale networks, including cortical and subcortical structures.