Ultrasonic imaging at 1,000 times times higher resolution
June 13, 2014
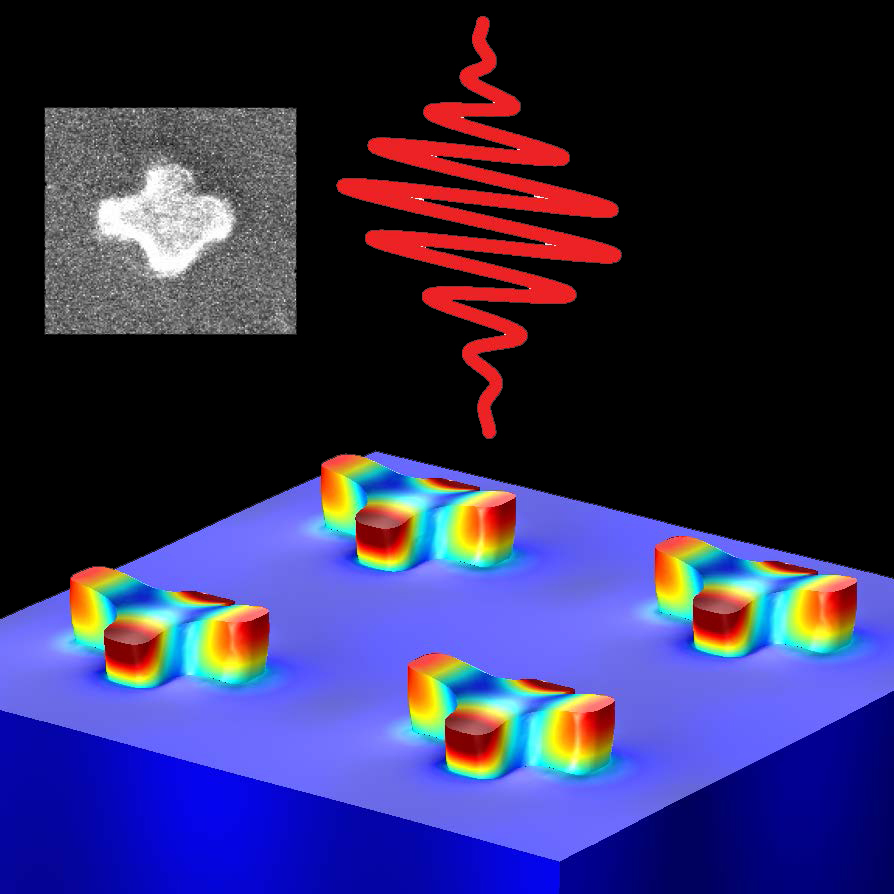
Gold plasmonic nanostructures shaped like Swiss crosses can convert laser light into ultrahigh frequency (10GHz) sound waves (credit: Berkeley Lab)
A next-generation ultrasonic imaging system that could provide 1,000 times higher resolution than today’s medical ultrasound systems has been demonstrated by Lawrence Berkeley National Laboratory (Berkeley Lab) researchers.
The researchers used a combination of subpicosecond laser pulses and unique nanostructures to produce acoustic phonons — quasi-particles of vibrational energy that move through an atomic lattice as sound waves — at a frequency of 10 gigahertz (10 billion cycles per second).
By comparison, medical ultrasounds devices today typically reach a frequency of only about 20 megahertz (20 million cycles per second). The 10GHz phonons can be used to “see” subsurface structures in nanoscale systems that optical and electron microscopes cannot.
“We have demonstrated optical coherent manipulation and detection of the acoustic phonons in nanostructures that offer new possibilities in the development of coherent phonon sources and nano-phononic devices for chemical sensing, thermal energy management and communications,” said research team leader Xiang Zhang, a faculty scientist with Berkeley Lab’s Materials Sciences Division and corresponding author of a paper published in Nature Communications describing this research.
Acoustic imaging offers several advantages over optical imaging. The ability of sound waves to safely pass through biological tissue has made sonograms a popular medical diagnostic tool. Sound waves have also become a valuable tool for the non-destructive testing of materials. Phonons at GHz frequencies can pass through materials that are opaque to photons, the particles that carry light. Ultrahigh frequency phonons also travel at the small wavelengths that yield a sharper resolution in ultrasound imaging.
Plasmons convert photons to phonons
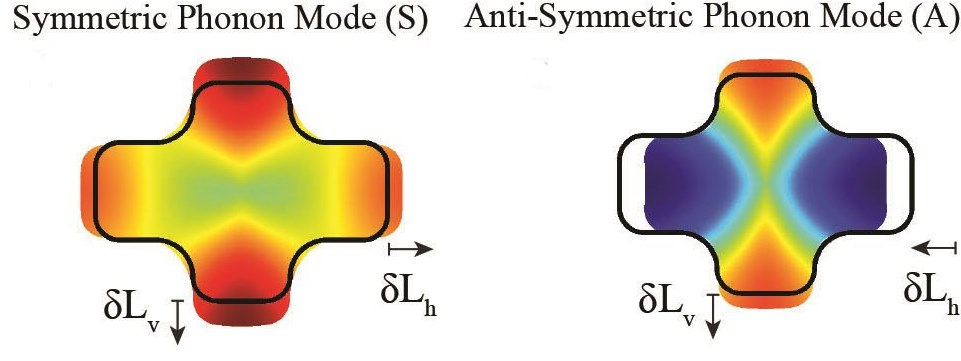
When the two arms of this Swiss-cross nanostructure oscillate in phase, symmetric phonons are produced. When the arms oscillate out of phase, anti-symmetric phonons are generated. The differences enable the detection of nanoscale motion. (Credit: Berkeley Lab)
But the biggest challenge has been to find effective ways of generating, detecting and controlling ultrahigh frequency sound waves. To meet this challenge, the researchers designed nanostructures that support multiple modes of both phonons and plasmons. A plasmon is a wave that rolls through the conduction electrons on the surface of a metal.
Plasmons can be used to confine light in subwavelength dimensions and are considered to be good candidates for manipulating nanoscale mechanical motion because of their large absorption cross-sections, subwavelength field localization, and high sensitivity to geometry and refractive index changes.
“To generate 10 GHz acoustic frequencies in our plasmonic nanostructures we use a technique known as picosecond ultrasonics,” said author are Kevin O’Brien. “Sub-picosecond pulses of laser light excite plasmons which dissipate their energy as heat. The nanostructure rapidly expands and generates coherent acoustic phonons. This process transduces photons from the laser into coherent phonons.”
To detect these coherent phonons, a second laser pulse is used to excite probe surface plasmons. As these plasmons move across the surface of the nanostructure, their resonance frequency shifts as the nanostructure geometry becomes distorted by the phonons. This enables the researchers to optically detect mechanical motion on the nanoscale.
The plasmonic nanostructures are made of gold and shaped like a Swiss cross. Each cross is 35 nanometers thick with horizontal and vertical arm lengths of 120 and 90 nanometers, respectively. When the two arms oscillate in phase, the crosses generate symmetric phonons. When the arms oscillate out of phase, anti-symmetric phonons are generated.
“The phase differences in the phonon modes produce an interference effect that allow us to distinguish between symmetric and anti-symmetric phonon modes using localized surface plasmons,” O’Brien says. “Being able to generate and detect phonon modes with different symmetries or spatial distributions in a structure improves our ability to detect nanoscale motion and is a step towards some potential applications of ultrahigh frequency acoustic phonons.”
By allowing researchers to selectively excite and detect GHz mechanical motion, the Swiss-cross design of the plasmonic nanostructures provides the control and sensing capabilities needed for ultrahigh frequency acoustic imaging.
For the material sciences, the acoustic vibrations can be used as nanoscale “hammers” to impose physical strains along different axes at ultrahigh frequencies. This strain can then be detected by observing the plasmonic response. Zhang and his research group are planning to use these nanoscale hammers to generate and detect ultrafast vibrations in other systems such as two-dimensional materials.
This research was supported by the DOE Office of Science through the Energy Frontier Research Center program.
Abstract of Nature Communications paper
Coherent acoustic phonons modulate optical, electronic and mechanical properties at ultrahigh frequencies and can be exploited for applications such as ultratrace chemical detection, ultrafast lasers and transducers. Owing to their large absorption cross-sections and high sensitivities, nanoplasmonic resonators are used to generate coherent phonons up to terahertz frequencies. Generating, detecting and controlling such ultrahigh frequency phonons has been a topic of intense research. Here we report that by designing plasmonic nanostructures exhibiting multimodal phonon interference, we can detect the spatial properties of complex phonon modes below the optical wavelength through the interplay between plasmons and phonons. This allows detection of complex nanomechanical dynamics by polarization-resolved transient absorption spectroscopy. Moreover, we demonstrate that the multiple vibrational states in nanostructures can be tailored by manipulating the geometry and dynamically selected by acousto-plasmonic coherent control. This allows enhancement, detection and coherent generation of tunable strains using surface plasmons.